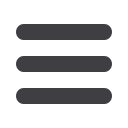
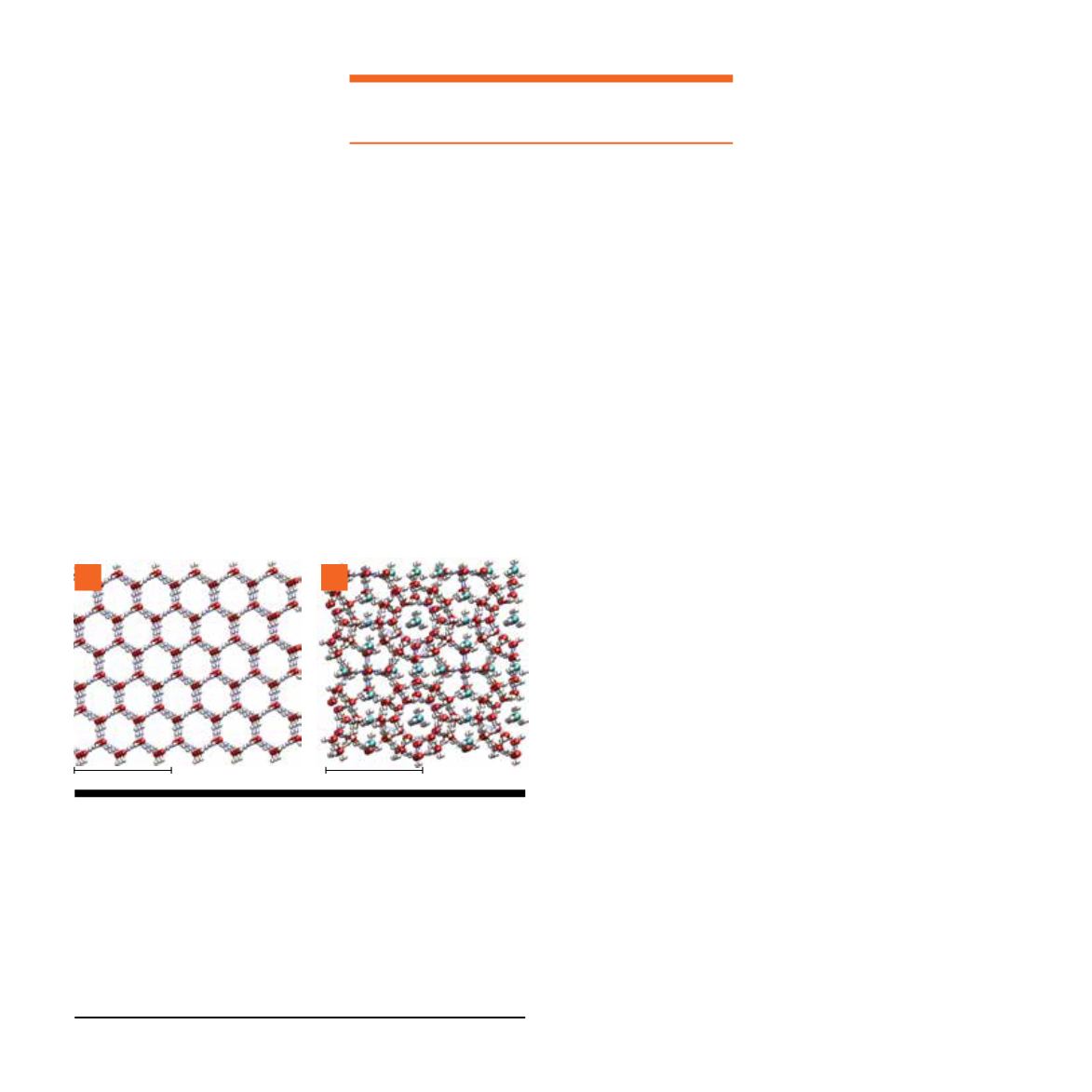
FROZEN HEAT
12
The English chemistry pioneer Sir Humphry Davy first com-
bined gas and water to produce a solid substance in his lab
in 1810. For more than a century after that landmark mo-
ment, a small number of scientists catalogued various solid
“hydrates” formed by combining water with an assortment
of gases and liquids. Sloan and Koh (2007) review this early
research, which was aimed at discerning the chemical struc-
tures of gas hydrates (Fig. 1.1), as well as the pressures and
temperatures at which they are stable. Because no practical
applications were found for these synthetic gas hydrates, they
remained an academic curiosity.
That perspective changed in 1934. Natural gas was begin-
ning to be used widely as a fuel and was often transported via
pipelines. Some pipelines were becoming plugged by what
appeared to be ice. E.G. Hammerschmidt (1934) discovered
the plugs were not ice, but gas hydrates. This initiated a wave
of engineering research – now known widely as flow assur-
ance – dedicated to predicting and preventing the formation
of hydrate blockages in industrial equipment. Since then,
as hydrocarbon exploration moved into deeper water where
hydrates form more readily, the oil and gas industry has in-
vested heavily in flow assurance research.
Much of the early gas hydrate research was empirical in nature,
as knowledge of the chemical structures of gas hydrates was still
limited. Determining the precise chemical formulation for gas
hydrates was challenging. A breakthrough came in the early
1950s, when a relatively new technology, X-ray diffraction, re-
vealed that gas hydrates were in fact clathrates, a term coined a
few years earlier to describe solids with no fixed chemical com-
position in which small guest molecules are trapped within a
host lattice. For many years, the combination of the predictive
power of a thermodynamic model for clathrate behaviour (van
der Waals and Platteeuw 1959) and crystal structure informa-
tion from X-ray diffraction provided the cornerstone of efforts
to predict gas hydrate properties based on their crystal structure
(Von Stackelberg and Muller 1951; Davidson 1973).
In the mid-1960s, following research into the pressures and
temperatures at which gas hydrates are stable (Pieroen 1955;
van der Waals and Platteeuw 1959), Y. Makogon and colleagues
in Russia recognized the natural association of methane and
water, and that the physical conditions (low temperatures and
high pressures) necessary to form gas hydrates should occur
naturally on Earth (Makogon 1965). In high-latitude perma-
frost regions, they predicted, gas hydrates should be found
starting hundreds of metres below the ground surface. In
marine environments, they should be found in shallow sea-
floor sediments beneath cold polar bottom waters where water
depths exceed approximately 300 metres, or in the sediments
beneath warmer, lower-latitude bottom waters where water
depths exceed 450-500 metres. Industry drilling in Arctic
permafrost confirmed the existence of naturally-occurring
gas hydrates in the early 1970s. It was not until a series of
1.1
INTRODUCTION
12Å
12Å
A
B
12Å
12Å
A
B
Figure 1.1:
Crystal structures of ice and methane gas hydrate. For
(A) ordinary hexagonal ice (ice Ih) and (B) structure I methane gas
hydrate (sI methane hydrate). Each sphere represents an atom:
white for hydrogen, red for oxygen, green for carbon. In hexagonal
ice, water molecules (H
2
O) are arranged in a hexagonal lattice. In
sI methane hydrate, water molecules form a lattice of cages, each
cage potentially holding a methane molecule (CH
4
). On the right
side of (B) and again toward the middle of (B), the cages line up,
appearing to hold a line of methane molecules (Figures courtesy B.
Anderson, WVU/NETL).
A
B