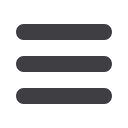

FROZEN HEAT
48
Geophysical methods hold great promise for the remote detection
and quantification of gas hydrate deposits because of the strong
changes in the physical properties that are induced by the presence
of gas hydrates. Replacing water or gas in the sediment pore space
with solid gas hydrates results in marked increases in the both the
electrical resistivity and the acoustic wave velocity of the sediment.
These physical changes can be detected with electromagnetic and
conventional reflection seismic technologies deployed from ships
and used to evaluate large regions. The data, when integrated
with models that correlate physical properties to gas hydrate
occurrence, make it possible to make general estimates of the
location, extent, and concentration of gas hydrate deposits prior
to drilling (Shelander
et al.
2010).
Box 2.3
Changing approaches to gas hydrate exploration
Direct detection of gas hydrate deposits, particularly those that are
widespread and highly concentrated, is a relatively new development in
gas hydrate science. Previously, the presence of gas hydrates in marine
sediments had been deduced seismically from the presence of a bottom-
simulating reflector (BSR; Shipley
et al.
1979), which commonly marks
the base of the gas hydrate stability zone (GHSZ). Physically, the BSR
is the transition from gas-hydrate-bearing sediments to gas-charged (or
at least gas-hydrate-free) sediments below. While early research focused
on how to exploit the seismic character of a BSR and infer gas hydrate
concentrations above and free gas concentrations below the reflection
(Hyndman and Spence 1992; Yuan
et al.
1996), more recent analyses
show that the seismic characteristics of BSRs cannot easily be related to
the concentration of the pore-filling material (Chapman
et al.
2002), a
conclusion confirmed by drilling results (Tsuji
et al.
2009).
A complementary technique, controlled-source electromagnetic
imaging (CSEM; Edwards 1997), attempts to exploit the increased
electrical resistivity of gas-hydrate-bearing sediments. However,
the physical nature of electromagnetic wave propagation through
marine sediments results in a reduced lateral and vertical
resolution, compared to seismic imaging. As a result, CSEM may
be more suitable for imaging chimney structures and other fracture-
dominated systems (Schwalenberg
et al.
2005).
Much prior gas hydrate exploration used sea-floor phenomena, such
as seabed hydratemounds, pockmarks, mud volcanoes, and depth of
sulphate penetration, as general indicators of the nature of historical
or current gas seepage. However, while these are interesting physical
features for understanding natural systems, they have not yet been
shown to be useful in prospecting for deeper reservoirs.
Recently, approaches to exploring for gas hydrate deposits have shifted
towards a more integrated evaluation of the full petroleum system
(Collett
et al.
2009). This approach incorporates geologic information
(such as the availability of gas sources, fluid migration pathways,
and suitable reservoirs) with direct geophysical indicators (such as
anomalous strong reflectors or high calculated velocities) in a way
regularly applied in the oil and gas industry (Saeki
et al.
2008 Boswell
and Saeki 2010). The approach acknowledges that all exploration
has great uncertainty, and that no single tool or piece of evidence
will be definitive and reliable. Instead, exploration uncertainty is best
managed by a comprehensive evaluation of all relevant data to provide
confidence in the occurrence of each necessary part of the system.
Figure TB-2.3:
A gas hydrate prospect delineated on the Alaska
North Slope. The image shows geophysically-inferred gas hydrate
trapped within a sand layer at the intersection of two fault planes
(green). (Courtesy US Geological Survey).
Bounding Fault
Below resolution
Approx. 1,500 m
Thickness and
gas hydrate concentration
increasing
Bounding
Fault
Approx. 3,000 m