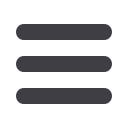

S180
ESTRO 36 2017
_______________________________________________________________________________________________
We conducted the neutron measurement under the
collaboration with National Institute of Standards and
Technology (NIST). We employed Bubble detectors (BTI,
Canada) to measure the neutron dose and energy
spectrum with good spatial resolution. The detectors
provide six energy thresholds from 10 keV to 10 MeV
allowing to validate dose and the neutron energy
spectrum. To simulate neutron scatter, a polyethylene
cylindrical phantom was milled and the bubble detectors
were placed inside. The phantom was then irradiated with
a Californium-252 neutron source to simulate the
secondary neutrons. We also simulated the experiment in
TOPAS to compute the neutron dose and energy spectrum
for comparison (Figure 1).
Results
The measured spectrum was unfolded and shows to be in
good agreement with the simulation. On average, the
percent difference in the spectrum was less than 31%
(Graph 1) and the percent difference of dose was under
23%. The agreement was best at the neutron energies 10
keV – 100 keV (19 %) and worst at 2.5-10 MeV (91 %). Better
statistics are needed for the higher energy spectrum
region. We plan to conduct the measurement three times
to minimize statistical errors and plan to extend the
validation to anthropomorphic physical phantoms.
Conclusion
We validated the dose and energy spectrum of scattered
neutrons computed from TOPAS Monte Carlo code by the
measurements using Bubble Detector. We plan to utilize
TOPAS dose calculation system coupled with patient-
specific proton therapy data for normal dose calculations
to support epidemiological studies of proton therapy
patients.
Proffered Papers: Treatment planning applications
OC-0345 Comparing cranio spinal irradiation planning
for photon and proton techniques at 15 European
centers
E. Seravalli
1
, M. Bosman
2
, G. Smyth
3
, C. Alapetite
4
, M.
Christiaens
5
, L. Gandola
6
, B. Hoeben
7
, G. Horan
8
, E.
Koutsouveli
9
, M. Kusters
10
, Y. Lassen
11
, S. Losa
4
, H.
Magelssen
12
, T. Marchant
13
, H. Mandeville
3
, F.
Oldenburger
14
, L. Padovani
15
, C. Paraskevopoulou
16
, B.
Rombi
17
, J. Visser
14
, G. Whitfield
13
, M. Schwarz
17
, A.
Vestergaard
18
, G.O. Janssens
19
1
UMC Utrecht, Department of Radiation Oncology,
Utrecht, The Netherlands
2
University Medical Center Utrecht, Radiotherapy,
Utrecht, The Netherlands
3
The Royal Marsden NHS Foundation Trust, Radiation
Oncology, Sutton, United Kingdom
4
Institute Curie, Radiation oncology, Paris, France
5
West German Proton Therapy Center Essen, Clinic for
Particle Therapy, Essen, Germany
6
Instituto nazionale dei tumori, radiation oncology,
Milano, Italy
7
Radboud university medical center, Department of
Radiation Oncology, Nijmegen, The Netherlands
8
Addenbrooke's Hospital, Radiation Oncology,
Cambridge, United Kingdom
9
Hygeia Hospital, Medical physics department, Athens,
Greece
10
Radboud university medical center, radiation oncology,
Nijmegen, The Netherlands
11
Aarhus University Hospital, radiation oncology, Aarhus,
Denmark
12
Oslo University Hospital, Radiation oncology, Oslo,
Norway
13
The Christie NHS Foundation Trust, Radiation oncology,
Manchester, United Kingdom
14
AMC, radiation oncology, Amsterdam, The Netherlands
15
Timone hospital, radiation oncology, Marseille, France
16
Hygeia Hospital, MEidcal Physics, Athens, Greece
17
Santa Chiara Hospital, Proton therapy Center, Trento,
Italy
18
Aarhus University Hospital, Medical Physics, Aarhus,
Denmark
19
University Medical Center Utrecht, Radiation Oncology,
Utrecht, The Netherlands
Purpose or Objective
The craniospinal irradiation (CSI) is challenging due to the
long target volume and the need of field junctions. The
conventional 3D-CRT technique (two lateral opposed
cranial fields matched to a posterior field) is still widely
adopted. Modern techniques (MT) like IMRT, VMAT,
Tomotherapy and proton pencil beam (PBS) are used in a
limited number of centres.
A multicentre dosimetric analysis of five techniques for CSI
is performed using the same patient, set of delineations
and dose prescription. We aimed to address two questions:
Is the use of 3D-CRT still justifiable in the modern
radiotherapy era? Is one technique superior?
Material and Methods
One 14 year-old patient with medulloblastoma underwent
a CT-simulation in supine position. The CTV and OARs were
delineated in one centre. A margin for PTV was added to
CTV: 5 mm around the brain and spinal levels C1-L2, 8 mm
for levels L3-S3. Fifteen SIOP-E linked institutes, applying
3D-CRT, IMRT, VMAT, Tomotherapy, or PBS (three centres
per technique), were asked to return the best plan
applicable for their technique: high weighting for PTV
coverage (at least 95% of PTV should receive 95% of the
prescribed dose) and low weighting for OAR sparing. Plans
for a prescription dose of 36 Gy were compared within and
between techniques, using a number of dose metrics:
Paddick conformity (range 0-1, with 1 being highly
conformal), and heterogeneity (range 0-1, with 1 being
highly heterogeneous) indices for brain and spine PTVs,
OAR mean doses and non-PTV integral doses.
Results
Conformity- (range 0.75-0.90) and homogeneity (range
0.06-0.08) indices of brain PTV were similar among all
techniques. However for the spinal PTV inferior indices
(CI: 0.30 vs 0.61 HI: 0.18 vs 0.08) are observed for 3D-CRT
with respect to modern techniques (Figure 1). Compared
to more advanced photon techniques, 3D-CRT increased
mean dose to the heart (13Gy vs 8Gy), thyroid (28Gy vs
15Gy), and pancreas (17Gy vs 12Gy) but decreased dose to
both kidneys (4Gy vs 6Gy) and lungs (6Gy vs 8Gy) (Figure
2). PBS reduced the mean dose to the OARs compared to
all photon techniques: a decrease of more than 10Gy was
found for parotid glands, thyroid and pancreas; between
5-10Gy for lenses, submandibular glands, larynx, heart,