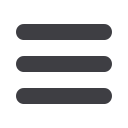

orientations may be found. Thus, a 3D reconstruction can be
generated by determining the orientations of individual par-
ticles. Single-particle approaches have been applied to heli-
cal structures
( 16), overcoming the need in the original
DeRosier and Klug method
( 14) for long-range helical order
in a structure to achieve a reasonable resolution. In a crystal,
long-range order is maintained by space group symmetry
but a helical polymer does not maintain such long-range or-
der. Deviations from an ideal or average symmetry accumu-
late, and a segment that is far from a reference segment may
show little correlation in terms of the expected positions of
subunits. This is similar to the bending of a polymer in so-
lution or a strand of spaghetti in boiling water. The confor-
mation of a polymer having the minimum energy is
typically a straight rod, but thermal fluctuations accumulate,
thereby knowing the orientation of the polymer at one point
does not allow one to predict the orientation of a segment a
long distance away. The helical viruses shown in
Fig. 2are rather rigid compared to flexible filamentous plant vi-
ruses, such as bamboo mosaic virus
( 17), but an analysis
of the helical symmetry in such viruses shows that long-
range helical order does not exist. With single particle
methods in cryo-EM, a reconstruction at ~3.8 A˚ resolution
( Fig. 3 )was obtained for
Sulfolobus islandicus
rod-shaped
virus 2 (SIRV2)
( 18), something that would not have been
possible at any reasonable resolution just 3 to 4 years
ago, before direct electron detectors were commercially
available.
The number of single particles used in 3D reconstructions
by cryo-EM can easily exceed hundreds of thousands, some-
thing that would have been computationally impossible back
in 1968 when 3D EM began. Just as progress in DNA
sequencing (due to the highly computational nature of cur-
rent sequencing) has roughly followed Moore’s Law, stating
that the number of transistors in an integrated circuit doubles
every 2 years, progress in cryo-EM has also followed the ad-
vances in computing power. Many images are needed to over-
come the inherently poor signal/noise ratio in cryo-EM.
Fig. 2shows an example of a typical micrograph. Most of
the power in the image, which is proportional to the variance,
or the sum of the squared deviations from the average inten-
sity, in the image is actually due to noise. A significant part of
this noise arises from the electron counting statistics or shot
noise because a low enough electron dose is used, which can
cause random fluctuations in electron counts in neighboring
pixels to become significant. This noise is more negligible as
many particle images are aligned and averaged together, and
a much greater problem becomes the conformational hetero-
geneity that exists for many molecules and complexes when
they are not packed tightly in a crystal.
The approach to this heterogeneity in solution NMR is to
generate an ensemble of structures, all consistent with the
constraints observed, whereas in cryo-EMonemight generate
a few structures that clearly correspond to different conforma-
tional states or only a single structure, excluding those parti-
cles that do not match this structure. For the ribosome, a recent
study
( 19) described starting with
>
1.6 million particles,
which were reduced to a more homogeneous subset of
~400,000 particles, yielding a resolution of better than
2.9 A˚ . We simply do not know the limit of resolution obtain-
able by cryo-EM for macromolecules. A recent structure
( 20)
of a bacterial enzyme,
b
-galactosidase, reached 2.2 A˚
( Fig. 4)
FIGURE 2 A cryo-EM showing two
Sulfolobus islandicus
rod-shaped
virus 2 (SIRV2) virions
( 18). SIRV2 is a virus that infects a hyperthermophile
living in nearly boiling acid. Almost all of the long capsid seen is formed by
several thousand identical copies of a coat protein that wraps around and pro-
tects the DNA. The arrows point to double-stranded DNA that can be seen
emerging from the ends of the virions. The scale bar is 500 A˚ .
FIGURE 3 The interior of a 3D reconstruction from SIRV2
( 18). Most of
the capsid protein in the virus is found in the form of
a
-helices, and at a res-
olution of ~3.8 A˚ the pitch and right-handed twist of these
a
-helices are
readily visible in the density map (
transparent gray surface
). Every capsid
protein is identical, and the building block of this assembly is a symmetrical
dimer formed by two copies of the capsid protein. Thus, the yellow and
green helices come from two different subunits and form antiparallel heli-
ces because the polypeptide chains are oriented in opposite directions from
each. At the available resolution, bulky aromatic side chains can be visual-
ized (
arrows
). Because the sequence of the capsid protein is known, this al-
lows for an unambiguous threading of the sequence through these helices.
To see this figure in color, go online.
Biophysical Journal 110(5) 1008–1012
1010
Egelman